particle accelerator
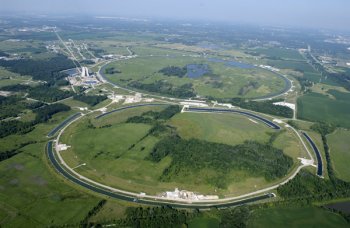
Figure 1. The particle accelerator complex at Fermilab, which included both a large synchrotron (the Tevatron) and a linear accelerator.. The Tevatron was shut down in 2011. Photo credit: Fermi National Accelerator Laboratory.
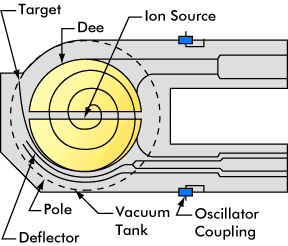
Figure 2. How a cyclotron works.
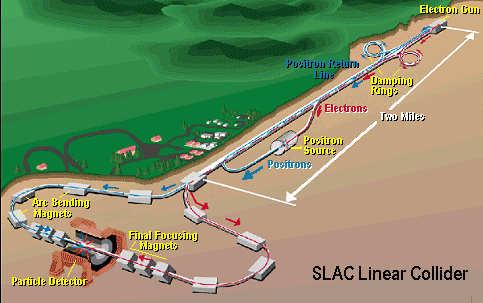
Figure 3. A linear accelerator: the Stanford Linear Collider.
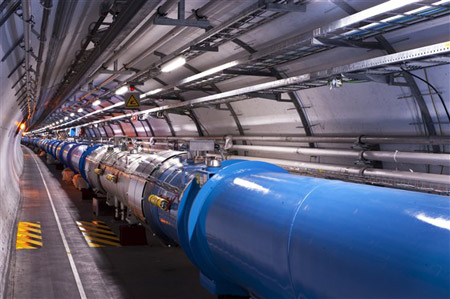
Figure 4. A section of the Large Hadron Collider showing the superconductor magnets.
A particle accelerator is a ring-shaped or linear (straight-line) device (Figure 1) that accelerates charged particles to high velocities and energies and, through the collision of these particles, allows the study of matter at very small scales (less than 10–12 centimeter). Modern accelerators can produce collisions that mimic the conditions of the early universe. Creating tiny fireballs of high density and high temperature, physicists are able to produce the particles that were abundant in the early universe, a trillionth of a second after the Big Bang. The largest accelerator in the world is the Large Hadron Collider near Geneva, on the border between Switzerland and France.
How accelerators work
An accelerator increases the velocity and hence the energy of charged particles through the use of alternating electric fields in an evacuated chamber. The particles must enter the high-frequency field as it begins increasing (negative particles) or decreasing (positive particles) in order to achieve the maximum energy increase. Magnetic fields are used to focus the particles into a narrow stable beam and to maintain the required curvature of the beam. As the particle velocity rise,s a relativistic increase in mass occurs.
In a linear accelerator, the particles travel in a straight line, usually accelerated by an electric field. In a cyclotron, particles are accelerated in a spiral path between pairs of D-shaped magnets with an alternating voltage between them. In a synchrotron, the accelerating voltage is synchronized with the time it takes the particle to make one revolution. A synchrotron consists of an (often very large) circular tube with magnets to deflect the particles in a curve and radio-frequency fields to accelerate them. The most advanced modern accelerators are colliders, in which beams of particles moving in opposite directions are allowed to collide with each other, thus achieving higher energies of interaction.
Types of accelerator
Betatron
The betatron was an early type of accelerator used specifically to accelerate electrons (beta particles) to energies of up to several hundred MeV. The electrons run in a evacuated, annular tube and are kept on this orbit by a magnetic field array. They are accelerated by electromagnetic induction (transformer principle).
Cyclotron
The cyclotron was conceived by Ernest O. Lawrence in 1929 as a way of obtaining high energy particles without having to use an extremely long linear accelerator. It was developed by Lawrence and his colleagues and students at the University of California in the 1930s.
In a cyclotron, charged particles such as protons or heavier ions are accelerated outwards in a spiral. Two large D-shaped dipole magnets produce a semicircular region of uniform magnetic field, pointing uniformly downward. The two D's are placed back-to-back with their straight sides parallel but slightly separated (Figure 2). An oscillating voltage is applied to produce an electric field across this gap. Particles injected into the magnetic field region of a D trace out a semicircular path until they reach the gap. The electric field in the gap then accelerates the particles as they pass across it. The particles now have higher energy so they follow a semicircular path in the next D with larger radius and so reach the gap again. The electric field frequency must be just right so that the direction of the field has reversed by their time of arrival at the gap. The field in the gap accelerates them and they enter the first D again. Thus the particles gain energy as they spiral around. The trick is that as they speed up, they trace a larger arc and so they always take the same time to reach the gap. This way a constant frequency electric field oscillation continues to always accelerate them across the gap. The limitation on the energy that can be reached in such a device depends on the size of the magnets that form the D's and the strength of their magnetic fields.
Once the synchrotron principle was developed, it was found to be a much cheaper way to achieve high energy particles than the cyclotron and so the original cyclotron method is no longer used in experimental high-energy particle research. Many cyclotrons, however, are still used around the world for the production of radionuclides for nuclear medicine, in particle therapy to treat cancer, and in low-energy particle physics experiments.
Linear accelerator
A linear accelerator, also known as a linac, is a type of particle accelerator in which charged particles travel in straight lines through a vacuum chamber (Figure 3). Early linear accelerators were electrostatic accelerators. In more modern types, a high-frequency, alternating electric field is used to accelerate the particles.
Final energies depend on the length of the chamber, and can reach several GeV (billion electron volts). The largest linear accelerator in the world, the Stanford Linear Accelerator (SLAC), was 3.2 kilometers (2 miles) long and produced electrons and positrons with energies up to 50 GeV. It was closed in 2008.
Synchrotron
A synchrotron is a circular accelerator (sometimes called a synchrocyclotron) which has an electromagnetic resonant cavity (or perhaps a few placed at regular intervals around the ring) to accelerate the particles (Figure 4). Particles pass through each cavity many times as they circulate around the ring, each time receiving a small acceleration, or increase in energy. When either the energy or the field strength changes so does the radius of the path of the particles. Thus, as the particles increase in energy the strength of the magnetic field that is used to steer them must be changed with each turn to keep the particles moving in the same ring. The change in magnetic field must be carefully synchronized to the change in energy or the beam will be lost. Hence the name "synchrotron". The range of energies over which particles can be accelerated in a single ring is determined by the range of field strength available with high precision from a particular set of magnets.
To reach high energies, physicists sometimes use a sequence of different size synchrotrons, each one feeding the next bigger one. Particles are often pre-accelerated before entering the first ring, using a small linear accelerator or other device.
Development of the accelerator
The earliest accelerators, called electrostatic accelerators, consisted simply of vacuum tubes in which electrons were accelerated by the voltage difference between two oppositely charged electrodes. The Van de Graaff generator and Cockcroft-Walton machine (see Cockcroft, John) work on the same principle but are larger and more elaborate. A further extension of the same idea is the linear accelerator, or linac, which is a sophisticated machine with many scientific and medical uses. All straight-line accelerators are handicapped by the finite length of the flight path, which limits the particle energies that can be achieved.
A major breakthrough in accelerator technology came in 1920 when Ernest O. Lawrence invented the cyclotron. In this device, magnets guide the particles along a spiral path, allowing a single electric field to apply many cycles of acceleration. Soon unprecedented energies were achieved, and the steady improvement of Lawrence's simple machine has led to today's proton synchrotrons, whose endless circular flight paths allow protons to gain huge energies by passing millions of times through the electric fields that accelerate them.
Until the 1960s, all accelerators were fixed-target machines, in which the speeding particle beam was made to hit a stationary target of some chosen substance. But then physicists began to build colliders, in which two carefully controlled beams are made to smash into each other at a chosen point. Colliders are more demanding to build, but the effort is well worthwhile. In a fixed-target machine, most of the projectile particles continue the forward motion with the debris after impact on the target. In a collider, on the other hand, two particles of equal energy coming together have no net motion, and collision makes all their energy available for new reactions and the creation of new particles.
The physics of accelerators
The beam in an accelerator, once established, is directed on to a target so that interactions may be produced. In a fixed-target experiment the target is stationary in the laboratory. Although historically of great importance, the main disadvantage of this type of experiment is apparent with the need to work at higher center-of-mass energies. The center-of-mass energy is important because it is a measure of the energy available to create new particles. In the laboratory frame at least some of the final-state particles must be in motion to conserve momentum. Consequently, at least some of the initial beam energy must reappear as kinetic energy of the final-state particles, and is therefore unavailable for particle production. The center-of-mass energy ECM is given in terms of the projectile's laboratory energy EL, by
![]() |
where mb and mt are the masses of the beam and target particles, respectively. At high energies this increases only like EL½ and so a decreasing fraction of the beam energy is available for particle production, the rest going to impart kinetic energy to the target. In contrast, if we could work in the center-of-mass system, all the available energy would in principle be available for particle production. This is achieved in a colliding-beam experiment, where two beams of particles traveling in almost opposite directions are made to collide at a small, or zero, crossing angle. If for simplicity we assume the particles in the two beams have the same mass and laboratory energy and collide at a zero crossing angle, then the total center-of-mass energy is
![]() |
This increases linearly with the energy of the accelerated particles, and is a significant improvement on the fixed-target result. Colliding beam experiments are not, however, without their own disadvantages: the colliding particles have to be stable, which limits the interactions that can be studied, and the reaction rates in such collisions are far smaller than those achieved in fixed-target experiments, because the particle densities in the beams are low compared to a solid or liquid target.
The rate Wr at which any particular reaction r occurs is proportional to the partial cross-section σ, which depends on the collision energy and the identities of the interacting particles. The constant of proportionality is called the luminosity L, that is
![]() |
where the luminosity contains all the dependencies on the densities and geometries of the beam and target. In a collider, where the target is one of the accelerated beams, the luminosity therefore characterizes the accelerator. A related quantity is the integrated luminosity LI, which is the integral of L over time, that is
![]() |
Finally, details of the particles produced in the collision (e.g. their energies and angular distributions) are deduced by observing their interactions with the material of detectors, which are placed in the vicinity of the interaction region. A wide range of detectors is available. Some have a very specific characteristics; others serve more than one purpose. Modern experiments in particle physics typically use several types in a single experiment.
Individual detectors are combined into modern complex detector systems. Detector systems have been used in non-accelerator experiments. The latter have been important historically and are becoming increasingly so again, particularly in the field of neutrino physics.