dark matter
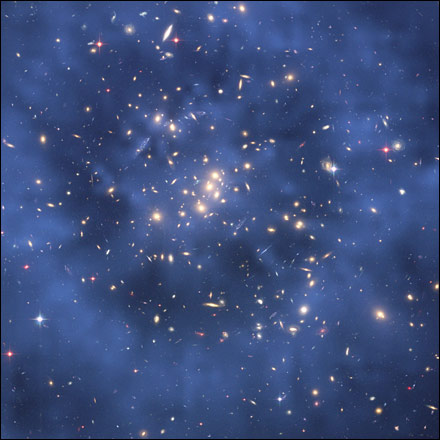
Figure 1. Hubble Space Telescope composite image showing what may be a ring of dark matter in the galaxy cluster Cl 0024 17. Image: NASA, ESA, M. J. Jee and H. Ford (Johns Hopkins University).
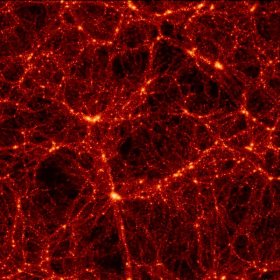
Figure 2. The distribution of dark matter obtained from a large numerical simulation. The dark matter is clustered into dark matter halos, which are connected by a large filamentary network. This simulation was performed by the Virgo consortium, including scientists from the Max-Planck Institute for Astrophysics (Jenkins et al. 1998, Astrophysical Journal, 499, 20-40).
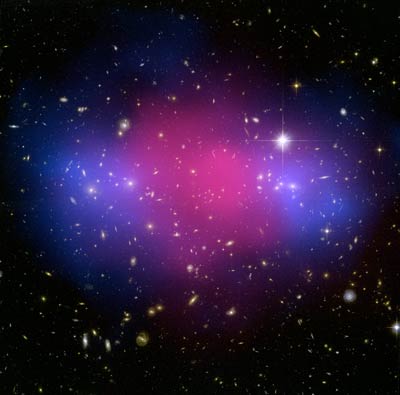
Figure 3. Cluster MACS J0025.4-1222.
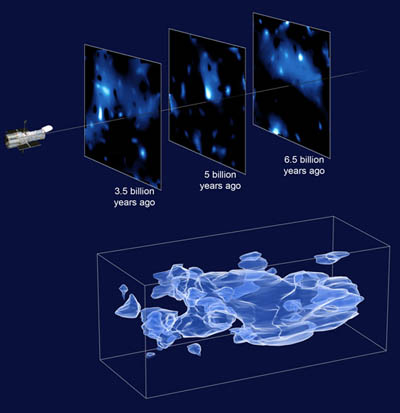
Figure 4. 3D distribution of dark matter.
Dark matter is matter that can't be detected directly, but whose existence can be inferred on the basis of how objects that we can see, such as stars and galaxies, move. Within a spiral galaxy like our own Milky Way, the stars move as if large quantities of dark matter exist in a vast halo that surrounds the galaxy's disk. Similarly, within clusters of galaxies, the individual galaxies move as if many times more matter is present than that visible in the form of stars and interstellar gas and dust.
Although the nature of dark matter remains uncertain, results published in February 2006 based on a study of 12 nearby dwarf galaxies (latest research section below) for the first time put numbers to some of its physical properties. This research showed that dark matter comes in "magic volumes", or standard packages, about 1,000 light-years (300 parsecs) across and containing about 30 million solar masses of material. Apparently, dark matter can't be crammed into spaces that are smaller than this. Another surprise is that the particles of which dark matter is made are moving on average with a speed of about 9 km/s, corresponding to a temperature of about 10,000 K – much higher than expected. This suggests that dark matter is neither hot nor cold but somewhere in between.
In addition to dark matter, the universe contains large amounts of another invisible ingredient known as dark energy. Overall, the cosmos seems to consist of 4% ordinary matter (mostly in the form of hydrogen and helium), 23% dark matter, and 73% dark energy.
Discovery
In 1932 Dutch astronomer Jan Oort turned his telescope toward visible stars in the disk of our galaxy and also began to observe the orbital velocities of stars within distant clusters of galaxies. In each case the stars that Oort observed appeared to be travelling too fast considering the combined gravitational pull from the systems in which they were embedded. He concluded that there had to be some form of hidden gravitational force dragging them onward – some kind of hidden matter.
The year after Oort's discovery, Swiss astronomer and physicist Fritz Zwicky correctly concluded, after examining the redshift in galaxies in the Coma Cluster, that the universe seemed to be made up mostly of what he called "missing mass." Zwicky, who mapped thousands of galaxies that presently make up the Zwicky Galaxy Database, was the first to measure evidence of unseen mass using the viral theorem of kinetic energy.
From measurements of how the galaxies were moving near the edge of the Coma Cluster, he came up with an estimate for the cluster's total mass which was 400 times greater than that expected based on the number of galaxies and the total brightness of the cluster. The gravitational tug of the visible matter in the cluster was nowhere near strong enough to explain the high speed of galaxies at the periphery. "[W]e arrive at the astonishing conclusion," said Zwicky, "that dark matter is present with a much greater density than luminous matter." Three years later, Sinclair Smith's observations of the Virgo Cluster revealed another huge mass discrepancy similar to that found by Zwicky.
Dark matter had been discovered. Yet this discovery was largely ignored at the time. Part of the reason was that scientists knew ways that observations like those of Zwicky and Smith could be misleading. Maybe a galaxy with a particularly high velocity isn't gravitationally bound to the cluster at all: it could be on its way out having exceeded the escape velocity, or perhaps it had never been part of the cluster, but was just sailing through. Maybe some of the observed high-speed galaxies lay in the foreground and just seemed to be part of the cluster because they fell along the same line of sight. Doubts like these kept Zwicky's dark matter off the scientific radar. However, the evidence quietly continued to build up.
In 1939, Horace Babcock looked at the rotation
of stars in the Andromeda Galaxy and concluded
that a lot of non-glowing matter had to be present in the outer parts to
explain the fast stellar motions there. Oort found the same kind of result in 1940 with another galaxy. In 1959 Franz
Kahn and Lo Woltjer studied movements within the Local
Group and showed that most of the mass in the Local Group is invisible.
Perhaps, they speculated, it took the form of very hot gas.
As the 1970s dawned, several researchers, including Vera Rubin, an astronomer
working at the Carnegie Institution's department of terrestrial magnetism,
looked in more detail at how stars rotated in Andromeda. By the middle of
the decade the conclusion that large amounts of unseen matter lurk in the
outskirts of galaxies was being supported by theoretical work on galactic
structure and stability. At last, the connection with the earlier work of
Zwicky and Smith was made and the problem of dark matter burst onto the
scientific stage. Most of the mass of galaxies, and therefore of the universe,
had apparently been overlooked.
Dark matter candidates
Some dark matter is familiar. Planets, moons, asteroids, and other minor debris, plus all the non-luminous gas and dust between the stars come into this category of ordinary dark matter. So, too, do all the very lightweight stars and so-called brown dwarfs that make up a lot of the stellar population of a galaxy like the Milky Way but contribute almost nothing to its brightness.
Part of all the dark matter that exists is bound to consist of familiar objects and materials like these. But there's very little chance it could be a significant part. The reason for this has to do with what happened in the Big Bang. At the birth of the universe, all that existed was a hot soup of subatomic particles. As the universe grew and cooled, the ordinary matter particles such as neutrons, protons, and electrons, started to join together to form atoms of the elements seen around the cosmos today – predominantly hydrogen and helium.
The theory of element-making in the first few minutes of the universe, called Big Bang nucleosynthesis (BBN), has been very successful. It not only predicts that hydrogen and helium should be dominant but it gets them in the right proportions we see, allowing for changes that have happened inside stars since. But with this success comes a catch. The amount of each element that forms depends sensitively on the amount of baryonic matter that the universe had available. Baryons are a family of heavyweight particles that includes the proton and neutron. BBN predicts the right ratios for the elements in the universe today only if the original amount of baryonic matter was no more than 10% of the critical amount of matter needed to stop the cosmic expansion. Because scientists believe dark matter makes up far more than 10% of the critical value, they're pretty confident that most dark matter isn't made of baryons.
It could be that, despite having worked so far, BBN will turn out to be flawed. But the prevailing view is that whatever dark matter is, it isn't made of baryons. Hence, it must consist of some kind of exotic matter, which is non-baryonic. Some non-baryonic dark matter might consist of a particle that's been known about for many years: the neutrino. Billions of neutrinos from space pass clean through the Earth every second. Although ghostlike, they're one of the most populous particles in the universe and, in recent years, it's been found that, contrary to previous belief, they almost certainly have a small amount of mass. It's possible, therefore, that they account for a significant portion of cosmic dark matter.
The other main non-baryonic dark matter candidate is called WIMPS (Weakly Interacting Massive Particles). These belong to a class of hypothetical heavy particles that hardly interact at all with familiar forms of matter. Examples of these particles, which have yet to be detected, include the axion and neutralino.
Mention should also be made of MACHOs (Massive Compact Halo Object) – the name chosen, in 1991, deliberately to contrast with WIMP. According to the MACHO point of view, sizeable galaxies like our own are cocooned by dark matter haloes that have a hefty population of non-luminous objects such as brown dwarfs, other types of very dim stars, black holes, planets and so on. Although we wouldn't be able to see MACHOs directly, we could, astronomers realized, hope to detect them in another way. Any concentration of matter can, under the right circumstances, act like a lens, bending and focusing the light rays from a source that lies behind them at a much greater distance. Since the early 1990s, a number of projects have been on the lookout for microlensing events that would give away the presence of MACHOs. Several extrasolar planets have been found by this technique. But, overall, not enough MACHOs have been found to account for more than a fifth of all dark matter, and the final figure may be much less. Also, because MACHOs are presumed to be made of ordinary (baryonic) matter, they're contribution is likely capped by the BBN restriction mentioned earlier.
Weighing the possibilities
Another way to categorize dark matter is into hot and cold varieties. Very light stuff that moves around at high speed is called hot dark matter (HDM); the top candidate for HDM is neutrinos. Particles of cold dark matter (CDM), by contrast, travel much more slowly; WIMPS are the chief suspect in this case. But does most dark matter run hot or cold?
Most theorists have favored the CDM model. But research published in 2011 on dwarf galaxies in the halo of the Milky Way, which are believed to consist mostly of dark matter, suggested that they can't form in the way they do if dark matter exists as CDM. The results of this research are, however, compatible with the notion of warm dark matter (WDM) which may have formed not within a millionth of a second after the Big, as in the case of CDM, but something like several minutes later. When simulations of galaxy formation are run with the later-forming WDM instead of CDM, the halo of dwarf galaxies has the same structure as is observed in reality.
The trouble is, if all dark matter is WDM and not CDM, then detecting it poses scientists with a problem. WDM would not show up, for example, in the Large Hadron Collider, an instrument that might have found CDM. Other possibilities exist for trying to detect WDM. The most likely WDM particle, the "sterile neutrino", could be identified by the X-rays it emits; but much more sensitive X-ray detectors would be needed.
Whatever dark matter is, it's the primary source of gravitation in the universe; so it must have played a crucial role in determining the structure of everything from galaxies to the large scale arrangement of superclusters (clusters of clusters) of galaxies. As theories, both CDM and HDM have their problems. It's hard to see how HDM could form lumpy structures such as galaxies and galaxy clusters. On the other hand, CDM has problems forming the biggest structures of all, like the vast walls along which astronomers have found superclusters of galaxies to be arranged. Until recently, the most popular view was that CDM accounts for the bulk of dark matter in the universe, with perhaps a minor contribution of 10 to 20% from HDM. A key prediction of the CDM model, that large galaxies are accompanied by huge retinues of dwarf galaxies (gravitationally drawn in by the CDM halos), won observational support in 2002. A study of large galaxies that act as gravitational lenses revealed that about 2% of their mass must be in the form of dwarf galaxies – equivalent to thousands of these small systems – to explain the lensing effects. Then, in 2006, was presented evidence that dark matter is of intermediate temperature.
Recent research
A detailed study of 12 dwarf galaxies that skirt the edge of our own Milky Way carried out by astronomers at the Institute of Astronomy, Cambridge, has pinned down some of the properties of dark matter. The study traced the dark matter in these dwarf systems by plotting the movement of their stars. With the aid of 7,000 separate measurements, the researchers established that the galaxies contained about 400 times the amount of dark matter as they do normal matter. They also found that the dark matter has a 'warm' temperature of some 10,000 K and is packaged into standard volumes. Further research will be needed to follow through the implications of this work.An intriguing prediction of theories about the Big Bang and the large-scale structure of the cosmos is that "dark galaxies" – galaxies made almost entirely of dark matter – should be very common. These starless objects would have formed from pockets of pure dark matter that are expected to have survived from the earliest days of the universe. In 2005, astronomers in the United Kingdom announced the discovery of the first dark galaxy, VIRGOHI21, lying 50 million light years away in the Virgo Cluster. Radio observations of the rotation of hydrogen gas in VIRGOHI21 have revealed there must be about a thousand times as much dark matter as hydrogen in this galaxy, and that its total mass is about one-tenth that of the Milky Way.
In 2007, researchers from Johns Hopkins University and the Space Telescope Science Institute, both in Baltimore, reported on their discovery of an extraordinary, vast ring uncovered they were mapping the dark matter distribution within a galaxy cluster known as Cl 0024+17. An image of this ring is shown at the top of this article. The cluster Cl 0024+17 lies 5 billion light-years away and the ring, apparently made of dark matter, is 2.6 million light-years across. The dark matter ring, which is thought to have been created following the collision of two galaxy clusters, acts as a gravitational lens, enabling astronomers to map the distorted light to deduce the cluster's mass and how dark matter is distributed in it.
Other recent discoveries bearing on dark matter
1. A powerful collision of galaxy clusters was captured by NASA's Hubble Space Telescope and Chandra X-ray Observatory (Figure 3). The observations of the cluster known as MACS J0025.4-1222 indicate that a titanic collision has separated the dark from ordinary matter and provide an independent confirmation of a similar effect detected previously in a target dubbed the Bullet Cluster. These new results show that the Bullet Cluster is not an anomalous case.
2. This three-dimensional map in Figure 4 offers a first look at the large-scale distribution of dark matter. This milestone takes astronomers from inference to direct observation of dark matter's influence in the universe. Because of the finite speed of light, regions furthest away are also seen as they existed a long time ago. The map stretches halfway back in time to the beginning of the universe.
The map reveals a loose network of dark matter filaments, gradually collapsing under the pull of gravity, and growing clumpier over time. This confirms theories of how structure formed in our evolving universe, which has transitioned from a comparatively smooth distribution of matter at the time of the Big Bang. The dark matter filaments began to form first and provided an underlying scaffolding for the subsequent construction of stars and galaxies from ordinary matter. Without dark matter, there would have been insufficient mass in the universe for structures to collapse and galaxies to form.
Top: Three slices through the evolving distribution of dark matter. The dataset was created by splitting the background source galaxy population into discrete epochs of time, looking back into the past. This is calibrated by measuring the cosmological redshift of the lensing galaxies used to map the dark matter distribution, and binning them into different time/distance slices. Each panel represents an area of sky nine times the angular diameter of the full Moon. Note that this fixed angle means that the survey volume is a really a cone, and that the physical area of the slices increases (from 19 megaparsecs (Mpc) on a side to 31 Mpc on a side) from left to right.
Bottom: When the slices across the universe and back into time are combined, they make a three-dimensional map of dark matter in the universe. The three axes of the box correspond to sky position (in right ascension and declination), and distance from Earth increasing from left to right (as measured by cosmological redshift). Note how the clumping of the dark matter becomes more pronounced, moving right to left across the volume map, from the early universe to the more recent universe.
The dark matter distribution was mapped with Hubble Space Telescope's largest ever survey of the universe, the Cosmic Evolution Survey (COSMOS). To compile the COSMOS survey, Hubble photographed 575 adjacent and slightly overlapping views of the universe using the Advanced Camera for Surveys' (ACS) Wide Field Camera onboard Hubble. It took nearly 1,000 houra of observations. The distances to the galaxies were determined from their spectral redshifts, using the Subaru telescope in Hawaii.